Elias Most, an assistant professor of theoretical astrophysics, did not want to be an astronomer or a physicist when he grew up. He studied physics but was more interested in computers; he even remembers buying a book about nonscience career paths for physicists, thinking he might end up in finance. His mind began to change in graduate school as he became interested in gravitational waves—ripples in the fabric of space-time generated by, among other violent events, the collisions of black holes. Then, in 2015, the Laser Interferometer Gravitational-wave Observatory (LIGO) made the first-ever direct detection of gravitational waves in space, and Most knew he was hooked.
Since then, Most has become an expert at simulating collisions between pairs of black holes as well as between pairs of neutron stars, both of which produce gravitational waves. When the most massive stars explode, or go supernova, they leave behind black holes; when stars that are a bit less massive but still heftier than our Sun explode, they leave behind neutron stars. "Neutron stars are the little sisters of black holes," Most says. "One major way in which they are different is that neutron stars are squishy. They are big blobs of squishy space goop."
Little is known about what happens when two massive neutron stars collide. While astronomers do know that neutron star collisions forge and disseminate heavy elements such as gold and platinum in dramatic explosions called kilonova, little is known about the fundamental physics behind these mergers. Most and his colleagues use supercomputers to simulate neutron star collisions and probe their hidden physics.
Most, who grew up in Germany, received his bachelor's degree from the University of Göttingen in 2013, and his PhD from Goethe University Frankfurt in 2020. He was a predoctoral fellow at the Flatiron Institute in New York from 2019 to 2020, and he held a joint postdoctoral appointment at the Institute for Advanced Study and at Princeton University from 2020 to 2023. He joined Caltech as a visiting associate in theoretical astrophysics in 2022 and became an assistant professor in 2023.
We sat down with Most to learn more about his background and ongoing quest to simulate astrophysical phenomena from the ground up.
Did you like physics growing up?
I liked it a little bit but nothing out of the ordinary. I think the coolest thing I did in high school was to participate in an office chair racing competition, which involved a little physics. We actually built office chairs to ride downhill and made it on to a Japanese TV show.
I was, however, really into computers and started using one when I was 4 years old. Surprisingly, the way you had to interact with these old computers back then is not much different from what we are doing today with modern supercomputers.
Later, I became more interested in science and enrolled in a physics and math undergrad program at the University of Göttingen, where I first came into contact with Einstein's theory of relativity. In grad school, I wanted to learn more about the fundamental building blocks of nature, so I moved to the University of Frankfurt. They are major players in the field of nuclear physics and are involved in building the world's largest heavy ion collider, which will smash together gold atoms. Interestingly, the work I do now on the collision of two neutron stars is the cosmic analog to this collider. It's the same physics but on a cosmic scale, blown up by 18 orders of magnitude.
What were you working on when LIGO made its famous detection of gravitational waves in 2015?
I was in grad school and many of my colleagues were working on the Event Horizon Telescope at that time. There were only a few of us working on gravitational waves and neutron star mergers. Back then, we thought nothing that interesting would happen in our field and then that all changed.
What fascinates you about neutron stars?
One thing that has always fascinated me about neutron stars is that studying them involves the confluence of many typically compartmentalized fields of physics. Since these stars contain predominantly neutrons, you need nuclear physics. But they are almost as compact as black holes, which requires Einstein's theory of relativity. On top of that, they can power electromagnetic signals, which is plasma physics. There are few fields where you can talk to people from three different communities, sometimes even four if you consider that many of the theoretical models we use are actually developed by mathematicians!
What are some of the problems you are working on involving neutron star mergers?
I really like problems that connect different fields or require you to think a bit outside the box. The outcome of a neutron star collision can either be a short-lived neutron star or a black hole. One thing we have recently investigated is how neutron stars that formed from mergers differ from regular stars like our Sun when it comes to their magnetic fields. The Sun has a very active 11-year cycle, changing its magnetic field periodically. We found that in mergers, neutron stars can do this within a fraction of a second! This also has implications for the gamma-ray bursts they can power.
Another really cool question we are following up on involves ideas that were developed at Caltech in the 1980s. Basically, there are earthquakes on the neutron stars right before they merge. The neutron stars interact through tidal or gravitational forces and shatter. They are like hard-boiled eggs with shells that crack and release energy. This energy could send out a precursor gamma-ray burst just seconds before the neutron stars merge and release gravitational waves. You hear this idea mentioned quite a lot—a few Caltech postdocs wrote a very nice paper on this about 10 years ago—but it turns out that nobody has ever simulated this, so you see a lot of fluff in the literature. We just had a paper out with my Caltech colleague Katerina Chatziioannou [assistant professor of physics, William H. Hurt Scholar] and two of the students here, which demonstrated what could actually happen and what our observer colleagues should be looking for.
How do your simulations complement the observations astronomers make of neutron stars?
Through our simulations, we can make predictions, but the starting point is always the physics. It's more of a bottom-up approach rather than a top-down. We start from basic principles and see what that tells us. For the questions I'm looking at, we don't know what the answer is most of the time. To give some concrete examples, we have looked into the interaction of neutron star magnetic fields prior to their collisions. Our simulations revealed that this may power novel flashes of radio light that may be detectable with the upcoming DSA-2000 radio telescope, which is being developed at Caltech. And, it turns out that a similar phenomenon may recently have been observed in a galactic white dwarf binary system.
How is simulating neutron stars different from simulating black holes?
Twenty years ago, people figured out, after many, many decades, how to make black holes merge on a computer. After that, they had to figure out how to do this with enough precision to be used by LIGO researchers searching for gravitational waves generated by these mergers. The Simulating Extreme Spacetimes (SXS) Collaboration founded by my colleagues Kip Thorne and Saul Teukolsky [Robinson Professor of Theoretical Astrophysics] at Caltech and Cornell has been at the forefront of this quest.
Black holes are entirely governed by Einstein's general relativity equations. You only need to know their masses and spins, and you are ready to go. But with neutron stars, we have general relativity, magnetic fields, hydrodynamics, neutrinos, and other factors to simulate. For some of these things, we don't even know yet how to describe or model all aspects in a computer simulation, and we are looking at much longer time periods since we want to know what happens after the neutron stars merge and explode in a kilonova. This is something we can only simulate well now thanks to breakthroughs in computing.
What are these breakthroughs?
The hardware devices, the GPUs [graphics processing units], in next-generation supercomputers are 500 times faster than before. The government invested billions of dollars into the development of these exascale computers. We have been using the supercomputers in Lawrence Berkeley National Laboratory in California, and at Oak Ridge National Laboratory in Tennessee. The challenging simulations used to take about a month, and now we can do them in a matter of hours. This is a massive speedup and will enable us to tackle new problems we couldn't even dream of before. It feels like we've entered a new era. It's really amazing.
Can you give us another example of when you have used these computers?
Besides neutron stars, we have recently started to think about black holes too, but different than those observed by LIGO. With the recent discovery of a stochastic gravitational wave background by the NANOgrav [North American Nanohertz Observatory for Gravitational Waves] collaboration, there has been a lot of recent interest in how supermassive black hole binaries form, come closer together, potentially through the interaction with surrounding gas, and, ultimately, collide.
This process is very hard to study numerically, as you need to simulate hundreds of orbits, 10 times more than what you need to simulate LIGO gravitational-wave events, and now you also have all the gas dynamics on top, which go along with supermassive black hole collisions since the black holes are surrounded by disks of gas. Not unsurprisingly, a lot of our intuition and knowledge of binary black hole gas interaction comes from two-dimensional simulations, which are much cheaper to do and, crucially, lack many important physics aspects, such as magnetic fields.
Recent findings from cosmological simulations in Phil Hopkins' [Ira S. Bowen Professor of Theoretical Astrophysics] group at Caltech, show that realistic gaseous environments around supermassive black holes may have ultrastrong magnetic fields altering their feeding behavior. Inspired by this new scenario, we performed a fully three-dimensional simulation of a supermassive black hole binary embedded in a gaseous disk with strong magnetic fields on the Oak Ridge GPU computers. This may well be one of the biggest simulations ever done within the SXS collaboration. Our simulation discovered a new magnetically arrested accretion state of the binary supermassive black holes that seems to help them come closer together, and it provides new avenues for electromagnetic observations. Understanding this more will probably keep us busy for a while.
What made you want to come to Caltech?
There's a lot of high-energy astrophysics research going on here, especially at TAPIR (Theoretical Astrophysics Including Relativity and Cosmology). There's LIGO, and a long history of gravitational physics and numerical relativity, including the SXS collaboration of which I am a part. There are also all the observers here doing cutting-edge work across the electromagnetic spectrum. And, most importantly for me, there is always a lot of new things to learn and to get inspiration from. As I mentioned earlier, I really like learning from related concepts in adjacent fields, most recently planetary magnetospheres and protoplanetary disks. And Caltech's small size means that I can easily just walk down the hall or across campus to talk to people in related fields and disciplines. Also, the nature and mountains in Pasadena are just amazing. Pasadena as a town is very impressive, too.
Do you have any favorite TV shows you've been into lately?
As you can see from my figures on my shelf, I like Star Trek. The new series Strange New Worlds is so good. I really like that they stuck to the original recipe for what makes Star Trek work well. My favorite series is Next Generation. I always like exploring new worlds.
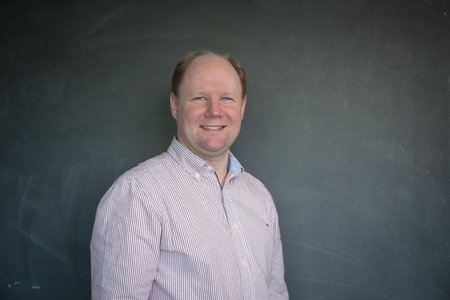
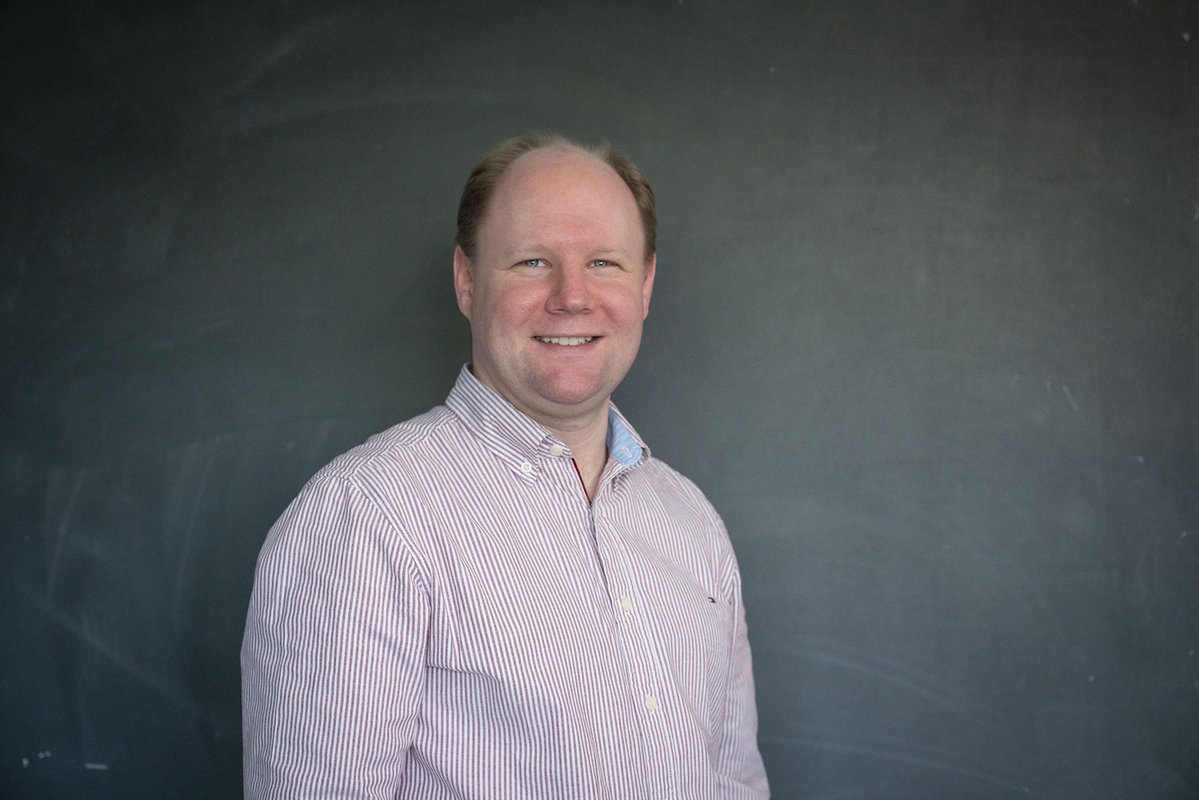